Abstract
Prostate cancer is among the primary causes of death among American men. It occurs due to the abnormal growth of prostate cells leading to the enlargement of the prostate gland. Various treatment methods are available to treat prostate cancer including surgery, cryosurgery, hormone replacement therapy, chemotherapy and watchful surveillance. However, despite the availability of all these techniques, many men continue to die from the disease. In addition, some of the adverse drug reactions and invasiveness of the treatment procedures affect the quality of life of prostate cancer patients. Recent developments have led to the use of nanotechnology in the treatment of prostate cancer. However, the technique is still under development. The aim of this study was to obtain evidence of the interaction between nanoparticles and the nuclei of cancer cells, particularly to elucidate the interactions between iron nanoparticles and the chromosomes of prostate cancer cells.
Iron oxide nanoparticles were synthesized by the co-precipitation of Fe2+ and Fe3+. Prostate cancer cells and normal cancer cells were incubated in a culture medium in the presence of varying concentrations of nanoparticles for 48 hours. The incorporation of the nanoparticles into the cells was determined using transmission electron microscopy. The effect of the nanoparticles on the prostate cells was established by determining cell viability after incubation. It was observed that cancer cells had a higher affinity for nanoparticles than normal cells and that the nanoparticles moved to the nuclei of the cells. The cytotoxicity of the nanoparticles was dependent on their concentration with concentrations of 50 x 1016 nanoparticles per mL having deleterious effects on prostate cancer cells. The cytotoxic effects were thought to be due to DNA damage and alteration of chromosomal structure.
Literature Review
Prostate Cancer
The prostate is a gland that is exclusive to the male reproductive system. It is situated just below the bladder and encircles the upper section of the urethra that conveys urine from the bladder. The main role of the prostate gland is to secrete certain fluids that contribute to the components of semen. The dimensions of the prostate gland vary with the maturity of an individual and have been reported to increase as the individual advances in age. Prostate cancer occurs when there is an overgrowth of the cells present in the prostate gland, which leads to the enlargement of the gland. Five forms of prostate cancer exist namely small cell sarcomas, adenocarcinomas, small cell carcinomas, transitional cell carcinomas and neuroendocrine tumors (Humphrey, 2012). Out of these, adenocarcinomas are the most common type of prostate cancer. The rate of development of prostate cancer varies among individuals and may be high or low.
The autopsy of several men who died from other diseases has revealed the presence of prostate cancer among these men, which implies that the disorder may be undiagnosed in some individuals due to a slow progression rate (Jahn, Giovannucci, & Stampfer, 2015). The American Cancer Society reports that one out of every seven men is at risk of developing prostate cancer in his lifetime (2016). The 2016 statistics in the United States indicate that 180,890 new cases of prostate cancer have been diagnosed so far and have resulted in the deaths of 26,120 people (American Cancer Society, 2016). Therefore, prostate cancer is the second most common cause of mortalities among American men after lung cancer.
Symptoms and Diagnosis of Prostate Cancer
Some of the indications of prostate cancer include difficulties passing urine, urinating more often than usual especially at night, blood-tinged urine or semen and erectile dysfunction. Metastasis of cancer to the bones may lead to hip, back and chest discomfort. Additionally, the enlarged cancerous cells may press onto the spinal cord leading to the loss of bladder and bowel control. The diagnosis of prostate cancer entails ascertaining the medical history of the patient and carrying out a physical examination. Some cases of prostate cancer are identified due to the manifestation of the disease symptoms. Usually, a blood test for the prostate-specific antigen and a digital rectal examination exam is performed. The findings of these tests then necessitate additional tests. For instance, the normal levels of the prostate-specific antigen are 4 nanograms per milliliter (ng/mL) of blood and any values above 4 are indicative of prostate cancer (Moyer, 2012). Some of the extra tests that are done to confirm the diagnosis of prostate cancer include transrectal ultrasounds or prostate biopsies. The findings of these tests are then used to define the cancer stage based on the histopathological findings. Five main stages of prostate cancer exist from stage 1 to 5 with stage 1 cancer having almost normal-looking prostate cells. On the other hand, stage 5 cancer has a large number of aberrant cells.
Treatment of Prostate Cancer
The treatment of prostate cancer consists of methods such as chemotherapy, surgery, cryosurgery, radiation therapy, hormone therapy, bone-directed therapy, vaccine therapy and watchful waiting where a patient’s symptoms are observed. Chemotherapy entails the oral or intravenous administration of anticancer drugs to target cancerous cells and is often useful in cases where cancer has metastasized to other parts of the body. Some of the commonly used chemotherapeutic agents include Mitoxantrone and Docetaxel (Beltran et al., 2011). However, unpleasant side effects may arise from these drugs including queasiness, vomiting, loss of appetite, hair loss, fatigue, and mouth sores. In addition, most of these drugs interfere with the composition of blood, particularly the concentration of leucocytes and platelets leading to increased susceptibility to infections and bleeding easily (Monsuez, Charniot, Vignat, & Artigou, 2010).
In prostate cancer, hormone therapy involves the reduction of the male sex hormones such as testosterone and dihydrotestosterone. The normal function of these hormones is to promote the growth of the prostate gland. However, such growth is undesirable for prostate cancer because it promotes the growth of cancerous cells alongside the normal prostate cells. Therefore, hormonal therapy, which is also referred to as androgen deprivation therapy (ADT) suppresses the concentrations of these hormones (Levine et al., 2010). Surgery is useful in cases where the disorder has not spread beyond the prostate gland. It entails the removal of the whole prostate gland as well the adjacent tissues through a process called radical prostatectomy. Cryosurgery, conversely, utilizes very cold temperatures to freeze and destroy malignant cells (Sciarra et al., 2012). This method is often used in the initial stages of cancer. Radiation treatment involves the use of high-energy radiation to destroy cancerous cells and may be used alongside hormonal therapy (Heidenreich et al., 2011).
Current Research on Prostate Cancer
Some of the shortcomings of the available prostate cancer treatment options include their invasiveness, inefficiencies, and unwanted side effects. To improve the prognosis of prostate cancer patients and mitigate some of the adverse reactions of the available treatment options, various aspects of the disease are being investigated including the genetics of prostate cancer, prompt detection techniques, staging of the disease, prevention, and advancement of novel treatment options. One new treatment for prostate cancer known as high-intensity focused ultrasound (HIFU) has been reported to eliminate malignant cells by using highly focused ultrasonic beams to heat up the cells (Pasticier, 2015). The employment of HIFU to cure prostate cancer has been used in countries such as Britain and China (Jang, Lee, Lee, Kim, & Hwang, 2010; Kennedy, Ter Haar, & Cranston, 2014). However, the technique has just been launched to the U.S. Studies are underway to establish its safety and efficacy before it can be fully embraced.
Genetic focus on prostate cancer seeks to understand the actual genes involved in the development of prostate cancer to design drugs that target these genes. For instance, a study by Shen and Abate-Shen (2010) has shown that telomere length plays a substantial role in the initiation and development of prostate cancer. Shen and Abate-Shen (2010) explain that the shortening of telomeres leads to DNA damage, which interferes with the stability of the chromosomes. Consequently, there is increased telomerase activity in most cancer cells thus implying that the length of telomeres is dynamically regulated in the advancement of prostate cancer. However, the automatic association between the reduction of telomeres and the instigation of cancer or stimulation of cellular senescence is currently unknown. Nevertheless, a number of approaches to control telomere length are being probed as probable therapeutic agents.
Magnetic Nanoparticles in Cancer Therapy
The use of nanoparticles in biomedicine has been proposed as an alternative method of drug delivery and treatment of cancer. One such form is hypothermia where cancerous cells are exposed to elevated temperatures. Cancerous cells have been reported to be more sensitive to temperatures above 41oC than normal cells (Roti Roti, 2008). Nevertheless, even normal cells are sensitive to extreme temperature changes to the sensitivity of enzymes to temperature changes. Therefore, the successful use of such a technique needs to be done in such a manner that protects normal cells from adverse temperature effects. Magnetically-mediated hypothermia generates heat through a vacillating magnetic field that takes advantage of magnetic nanoparticles as the heating centers.
MNPs provide several benefits the first of which is the nanoscale size of their heat probes that enable intravenous administration and conveyance to tumors through the bloodstream (Joshi, Tiwari, Tiwari, & Srivastava, 2012). They have a high surface area to volume ratio, which makes it possible to customize with several recognition molecules that can all be directed towards specified malignant tissues (Lu, Liong, Li, Zink, & Tamanoi, 2010). The third advantage is that distant heating of the magnetic nanoparticles by the superficially harnessed magnetic field permits the action of heat only in the areas where the nanoparticles have accumulated (Guardia et al., 2012). Additionally, the physical properties of iron nanoparticles have made them suitable for cancer therapy through in vitro or in vivo imaging, and magnetic drug directing (Gautier, Allard-Vannier, Munnier, Soucé, & Chourpa, 2013). However, the precise mechanisms of magnetic nanoparticles in destroying cancer cells through magnetic drug targeting have not been clearly elucidated. Several studies propose that the nanoparticles interact with the chromosomes of cancerous cells within the nucleus thus exerting their therapeutic properties. The aim of this study is to ascertain the type of interaction between cancer cells and iron oxide nanoparticles.
Transmission Electron Microscopy
Transmission electron microscopy is a microscopy method that utilizes a ray of electrons to generate images of the tiny specimen. A beam of electrons is passed through a thin layer of the sample of interest (Glauert & Lewis, 2014). As the electrons traverse the sample, they interact with the electrons present in the specimen leading to the generation of an image that is amplified and directed towards an imaging gadget. The imaging contrivances may be fluorescent screens, photographic films or cameras (Pennycook & Nellist, 2011). Due to their use of electrons as opposed to light, transmission electron microscopes are capable of producing high-resolution images that make it possible to visualize fine details that are unattainable with a light microscope. As a result, transmission electron microscopes form a vital part in various fields of research such as material science, nanotechnology, oncology, and virology (Reimer, 2013).
Hypothesis
It was hypothesized that iron oxide nanoparticles would interact with the chromosomes present in prostate cancer cells.
Objective
The main objective of this study was to obtain evidence of the interaction between nanoparticles and the nuclei of cancer cells.
Specific Objective
The specific objective of the study was to elucidate the interactions between iron nanoparticles and the chromosomes of prostate cancer cells.
Material and Methods
The Synthesis of Iron Oxide Nanoparticles
All methods illustrated in this paper were adapted from a Ph.D. dissertation “Characterization and evaluation of surface-modified superparamagnetic iron oxide nanoparticles for uptake into human prostate carcinoma cells” written by Nefertiti in 2011. Magnetite was utilized as the base material for the superparamagnetic iron oxide nanoparticles (SPION) in the form of non-coated MNPs. A stock solution of magnetite MNPs was synthesized in an aqueous solution, and aliquots were measured for the preparation of surface-modified magnetite nanoparticles. An aqueous mixture of FeCl2 and FeCl3 was added to a beaker containing ammonium hydroxide and water and mixed on a hotplate without heat (Nefertiti, 2011).
Non-Coated Magnetite Particles
SPIONs were synthesized by simultaneous precipitation of 2 parts of FeCl3 and 1 part of FeCl2 (Fischer Scientific Fairlawn, NJ). The iron salts and 20 mL of de-ionized water were mixed in Nalgene polycarbonate containers using a stir bar coated with Teflon for approximately 20 minutes until everything dissolved. A dilute solution of ammonium hydroxide solution containing 15 mL of NH4OH and 65 mL of de-ionized water was prepared in a similar container and stirred for five minutes. The mixture of iron salts was quickly added to the ammonium hydroxide solution. Thereafter, the alkaline solution was stirred at high speed for 60 minutes with the container covered to form naked magnetite nanoparticles. The resultant mixture had a deep brown to black color with a pH of 5.94. The solution was transferred into plastic 50 mL centrifuge tubes and ultra-centrifuged at 10,000 rpm for 10 minutes. The supernatant was poured out and thrown away. The pelleted nanoparticles were resuspended in fresh deionized water during a sonication step (Fisher Scientific, New Jersey, USA). Centrifugation and sonication were repeated until all chloride ions were removed from the nanoparticles. Maximum centrifugation speeds of 20,000 rpm for 1 hour at 10 oC were used in the final steps. A drop of one mole of silver nitrate was added to the supernatant from the ultracentrifugation of the nanoparticles to determine the presence of chloride ions. Centrifugation and sonication were repeated until no precipitate was formed. The pure particles were resuspended in ultrapure water and refrigerated at 4˚C.
Transmission Electron MicroscopyTechnique
A dilute solution of nanoparticle concentrations between 1 X 104 and 1 X 1017 nanoparticles per mL was dropped on the grid and allowed to dry at room temperature after which it was placed in the vacuum chamber of the TEM. Electrons were passed through the material of interest, which was then magnified and focused by an objective lens. The image was displayed on a monitor, photographed and printed for review.
The presence of magnetite nanoparticles in human prostate cells was also confirmed by TEM. The sample preparation involved discarding the treatment media from the samples after a 48-hour treatment period. Approximately 10 mL of FG fixative dissolved in 0.2 M sodium cacodylate buffer at pH 7.4 was added to each T75-flask containing the cells and fixed overnight. A rubber policeman was then used to scrap the fixed cells from the surface of the flask. The cells were collected into 15 mL centrifuge tubes and washed with 0.2M sodium cacodylate buffer followed by serial alcohol dehydration (concentrations of 35%, 50%, 75%, 95% and finally in 100% ethanol) 3 times for 10 minutes each. The specimen was then infiltrated using 1:1 spurs with ethanol for 1hr at room temperature followed by 100 % spurs for 1 hour before being placed in capsules and gestated for polymerization. A Leica microtome was used to obtain thick slices (490 nm) of the polymerized pellet followed by thin sections (90 microns) that were collected on copper grids and stained with uranyl acetate and lead citrate before observation using a Zeiss (Model 10CA) transmission electron microscope.
Preparation of Samples
Culturing of Normal Human Prostate Cells
Normal human prostate cells used were WPMY-1 (ATCC number CRL-2852) obtained from American Type Culture Collection (ATCC) Manassas, VA. The source of the cells was a 54-year-old Caucasian male. The maintenance of the cells was performed in complete RPMI containing 10% FBS and penicillin and streptomycin antibiotics (100,000 U/L and 100 mg/L) respectively. The cells were kept in a humidified incubator controlled at 37˚C and 5% CO2.
Culturing of Cancer Human Prostate Cells
Human prostate cancer cells used were DU 145 (ATCC number HTB-81) bought from ATCC (Manassas, VA). The source of the cells was a 69-year-old Caucasian male. The propagation and maintenance of the cells were done in the same medium and conditions used for the normal human prostate cells.
Cytotoxicity of Iron Oxide Nanoparticles
Culturing of Cells with Iron oxide Nanoparticles
Prostate cancer cells (du-145) and normal cells (WPMY-1) were plated in six wells containing various concentrations of magnetite nanoparticles for 48 hours. The concentrations of the MNPs were 0, 2.5, 5, 7.5, 10 and 50 x1016 MNPs/mL.
Directional Growth during Proliferation
Approximately 30,000 prostate carcinoma cells were planted in distinct single wells with diameters of 35 mm. The cultured cells reached 60% confluence within two days after which the conventional RPMI 1640 growth medium was substituted with media containing sodium citrate coated magnetite nanoparticles. About 100 μL of the nanoparticle stock solution was added to 9.9 mL of RPMI media. 3 mL of this reconstructed nanoparticle and media blend was added to the prostate cancer cells in culture. A neodymium magnet was put beneath the culture dish with the medium and cells for 48 hours. The cells were observed under a microscope.
MTT
The cytotoxic impact of the magnetite nanoparticles on prostate cancer and normal prostate cells was evaluated using MTT assay (ATCC#30-1010K). MTT is a chemical referred to as 3-(4,5-Dimethylthiazol-2-Yl)-2,5-Diphenyltetrazolium Bromide. The plasma membrane potential leads to the absorption of the chemical by cells, which then reduces it to formazan through the action of intracellular NAD(P)H-oxidoreductases. A microplate reader was used to measure the absorbance followed by the enumeration of the cells of interest. A 100 μL cell suspension produced 20,000 cells per well in a plate containing 96 wells. The cells were kept warm at 37˚C and 5% CO2 for 24 hours before being treated with various amounts of magnetite nanoparticles ranging from 0.5 x 1016 to 50 x 1016 particles per ml. The experimental setup utilized two controls, which were cells in the conventional culture media and culture media devoid of cells. The 96-well plate was gestated for 48 hours at 37˚C and 5% CO2 after which the cells were washed with 1X PBS before the addition of medium containing 10% (v/v) of 3mg/ml MTT as described by Darmani et al. (2006). The plates were further incubated for 3 to 4 hours before suctioning the media drying the plates overnight at room temperature. About 100 μL of a 0.04 N HCl in isopropanol solution (Storch et al., 2004) was introduced to each well and incubated at room temperature in the dark for 30 minutes. The purpose of the process was to liquefy the formazan crystals. Thereafter, the absorbance of the plates was read at a wavelength of 570 nm using a microplate reader.
Trypan Blue Exclusion
Trypan blue exclusion was performed to determine cell viability in a cell suspension that uses a blue dye. The cell suspension was centrifuged for 5 minutes at 100 x g, and the supernatant was discarded. The cell pellet was re-suspended in 1 mL of PBS. About 20 μL of the cells and 2 μL of the dye were mixed and kept warm at room temperature for 3 minutes. Thereafter, the number of cells in 10 μL of the mixture was determined on a hemocytometer. The total number of viable cells per mL of the aliquot was determined by finding the product of the sum of viable cells by the dilution factor for trypan blue (2). The total number of cells per mL of the aliquot, the total number of viable and nonviable cells were obtained and multiplied by 2. The percentage of viable cells was then computed using the formula:
Viable cells (%) = (total number of viable cells per mL of aliquot/total number of cells per mL of aliquot) x 100.
Results
Magnetic Properties of Magnetite Nanoparticles
The co-precipitation of Fe2+ and Fe3+ ions led to the formation of magnetic magnetite nanoparticles of various sizes. The average diameter of the small-size particles was 5 nm while the average diameter of the big-sized particles was 35nm. Additionally, the saturation magnetization for the non-coated iron MNP’s ranged from 60 to 70 emu/g. These observations were in line with the expected saturation magnetization for pure magnetite nanoparticles, which is usually approximately 70 emu/g and increases with an increase in the size of nanoparticles (Estephan, Hariri, & Schlenoff, 2013). The dispersion of the nanoparticles in water and medium yielded varying outcomes with bare, uncoated nanoparticles yielding the largest particle size of 645 nm.
At 400X magnification, the cells showed magnetic interaction and rapid motion while reacting to the presence of the neodymium magnet that was put approximately 1.25 inches from the central position in four distinct locations namely north, south, east, and west.
Dimensional Measurements of Prostate Cells
The length of normal and cancerous prostate cells cultured independently in standard T-75 cell culture flasks were measured using a light microscope’s 10X objective lens and screen calipers that had been calibrated with a sample of known size. The length of prostate cancer cells was 83.16 nm while that of the average normal prostate cell was 267.33 nm. Overall, the normal cells were two to threefold larger than the prostate cancer cells (Nefertiti, 2011).
Cellular Re-alignment in Response to Neodymium Magnet during Proliferation
Prostate carcinoma cells were left to divide and grow in the presence of MNPs. The reason for this process was that efficacious assimilation of nanoparticles into the cytoplasm of prostate cells would be demonstrated by a certain effect when a strong neodymium magnet was put beneath the culture dish containing the cells. The magnetic dealings between the nanoparticles and the magnet directed the cells and made them grow along with the directions of the two opposite poles. There were changes in the morphology of the cells in response to the magnetic nanoparticles and the guiding forces of the applied magnet. The cells’ response was as expected. However, it remained unknown whether the magnetic influence was exerted from the exterior or interior of the cell membrane.
Magnetic Responsiveness of Prostate Cells Containing Magnetite Nanoparticles
Normal and cancerous prostate cells that were cultured in media containing magnetite nanoparticles showed the capacity to incorporate the nanoparticles into their cytoplasm. Evidence of magnetic responsiveness was observed from a series of still images. One prostate cancer cell entered the field of view from the upper section when a neodymium magnet was placed on the right of the glass slide. The cell revolved stopped for a short when large-sized nanoparticles interacted with the magnetic field and pushed it to the left and out of the field of view.
Visual Verification of Nanoparticles within Prostate Cells by Transmission Electron Microscopy
The movement of MNPs from the outer to the inner section of the prostate cells was noted by transmission electron microscopy. The process began when nanoparticles contained in the nutrient media interacted with microvilli on the outer surface of the prostate cells. The nanoparticles were engulfed and given access into the cell through the process of endocytosis (Figure 1 image 1). Once they entered the cell, the nanoparticles were collected and pigeonholed in tiny vacuoles containing magnetic iron nanoparticles, which can be seen in Figure 1 image 2. The smaller vacuoles joined up to create larger vacuoles, which raised the number of pigeonholed nanoparticles thereby increasing the number of MNPs within these assemblies in the cell cytoplasm. Following the fusion of the smaller vacuoles into bigger vacuoles, the nanoparticles migrated towards the cell nucleus, where probable chromosomal interaction occurred.
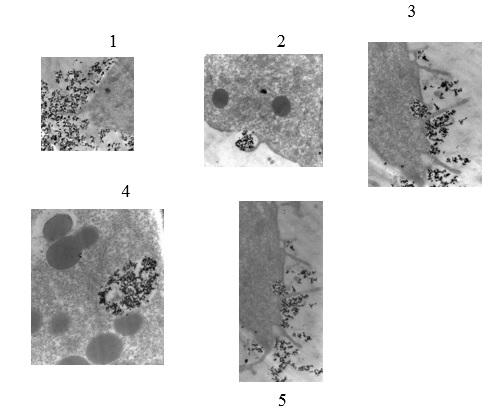
Scale: 1 cm represents 50 µm. The images were adapted from an old study carried out by Dr. Ayele Gugssa. In 1, the nanoparticles in the medium associate with microvilli on the outer surface of the prostate cells as indicated by the small dark dots surrounding the cell. 2 show the process of endocytosis as the nanoparticles are engulfed and enter the cell through the cell membrane. 3 shows the aggregation of nanoparticles into tiny vacuoles as indicated by small dark dots. 4 shows the movement of the large vacuole formed by the aggregation of smaller vacuoles towards the nucleus of the cell. It is noted that all nanoparticles in the cell are selectively moving towards the nucleus of the cell. In 5, the nanoparticles have traversed the nuclear membrane and are interacting with the DNA of the cancer cells. It is at this point that chromosomal interactions with nanoparticles occur.
Prostate Cell Viability after Exposure to Various Nanoparticle Concentrations
Cell counts with a hemocytometer following the removal of cells from the culture medium after the 48-hour treatment period showed the effect of nanoparticle concentration on the survival of cells. The highest nanoparticle concentration of 50 x 1016 nanoparticles/mL led to the death of prostate cells while nanoparticle concentrations ranging from 104 to 1015 were not strong enough to elicit magnetic properties and pose cytotoxic effects on the cells. Overall, the cytotoxic effects increased with an increase in the nanoparticle concentration as was evidenced by the increase in the number of dead cells with increasing MNP concentration. As the nanoparticle concentration per mL decreased, the cell survival increased. However, MTT or Trypan blue exclusion did not produce accurate results because of the adherence of cells in the culture flasks hence interfering with the enumeration of cells.
Discussion
The magnetic traits of the synthesized nanoparticle systems corresponded to the published values of magnetic nanoparticles (Santoyo Salazar et al., 2011). Physical interaction of cells with colloid-stable nanoparticles during incubation permitted the conveyance of magnetite nanoparticles from the outer part of the prostate cell through the cell membrane and into the cytosol as shown in 1 above. Thereafter, phagocytosis, where the cell membrane invaginated to collect particulate matter in a pocket and followed by pinching it off to engulf it occurred as indicated in image 2 in figure 1 above. Image 3 indicates the motion of the nanoparticles towards the nucleus of the cell in response to the applied magnetic field. A “call and response” liaison was seen when living cells became magnetically charged receptacles that were manipulated by magnets. One main distinction between normal and prostate carcinoma cells is the comparatively higher metabolic requirements of prostate carcinoma cells. This difference was accountable for the higher uptake of the nanoparticles by the prostate carcinoma cells than normal cells.
Prostate Cell Viability after Exposure to Various Nanoparticle Concentrations
Initial trials were performed with low concentrations of the synthesized magnetite nanoparticles. It was found that magnetite nanoparticles of concentrations ranging from 104 to 1015 were not adequately concentrated to produce magnetic property information. It was also observed that no cytotoxicity effects were present at these concentrations. Interestingly, prostate cells thrived, and their populations were compared to the normal cells. This observation was in line with various experiments that show that the concentration of nanoparticles is vital to the exertion of their cytotoxic effects (Naqvi et al., 2010; García et al., 2011; Laurent, Dutz, Häfeli, & Mahmoudi, 2011).
MTT findings were not consistent due to the discoloration of the walls of the plates by the nanoparticles thereby obstructing the spectrophotometric reading of the plate reader. The maximum nanoparticle concentration of 50 x 1016 nanoparticles/mL was deadly to prostate cells. The overall trend observed was that the survival rate of prostate cells decreased as the nanoparticle concentration per mL was increased and vice versa. The utilization of use of trypan blue for the elimination of blue-stained dead cells was inappropriate because the live prostate cells that were stained red made it difficult to tell apart the living and dead prostate cells.
Interaction of Nanoparticles with Chromosomes of Prostate Cancer Cells
It was observed that prostate cancer cells took up more nanoparticles than normal prostate cells. Additionally, the cell viability of the cancerous cells reduced as the concentration of the nanoparticles was increased. The TEM images also showed the accumulation of nanoparticles in the nucleus of cancer cells. These findings implied that the nanoparticles had an increased affinity for the cell nuclei. The fact that cancer cells died with an increase in the dosage of nanoparticles pointed towards interference with the structure and integrity of DNA, which was necessary for the survival of the cells. DNA controlled the various processes that were necessary for the replication of a cell. There was a likelihood that the nanoparticles affected the cell cycle of the cell by affecting chromosomes.
A study by Mahmoudi, Azadmanesh, Shokrgozar, Journeay, and Laurent (2011) has reported that nanoparticles affected the cell cycle of cells by associating with DNA. In their study, they investigated the precise effect of nanoparticles on DNA through a series of polymerase chain reactions. They reported that nanoparticles with a strong attraction for DNA constrained the duplication of DNA (Mahmoudi et al., 2011). This process was responsible for the observed toxicity effects of the nanoparticles. These findings corroborated those reported by Zhao et al. (2013) regarding the effects of DNA damage on the cell cycle. In their study, Zhao et al. (2013) interfered with the integrity of DNA by introducing mutations whose effects were inhibiting the replication of DNA and interrupting the progress of cells through the cell cycle thus triggering apoptosis. Interference with DNA replication in the initial stages of the cell cycle leads to apoptosis as confirmed by a separate study by Silva et al. (2011) who reported that magnetic hyperthermia of cancerous cells led to the death of the malignant cells through apoptosis.
The increase in temperature alters the operations of numerous enzymatic and structural proteins within the cells, which consequently inhibit the growth and differentiation of cells that trigger apoptosis. The cellular modifications brought about by hypothermia also alter transmembrane transport and subvert the membrane potential (Alvarez-Berríos et al., 2013; Franke, Kettering, Lange, Kaiser, & Hilger, 2013; Petryk, Giustini, Gottesman, Kaufman, & Hoopes, 2013). The elevated temperatures also interfere with the synthesis of nucleic acids and impair the action of repair enzymes, which stimulate changes in the arrangement and structure of DNA. As a result, the malformed DNA cannot function appropriately in directing the functions of the cell leading to cell death.
DNA is sensitive to temperature increases, which leads to its separation into single-stranded DNA. Additionally, hyperthermia increases a cell’s sensitivity to factors that hamper DNA metabolism thus indicating that hyperthermia plays a direct role in inhibiting the repair of DNA. Initial investigations report that hyperthermia favors the formation of disruptions in DNA as well as chromosomal abnormalities by denaturing proteins or impeding the replication of DNA. One possible mechanism is through elevated concentrations of 8-oxoguanine, loci lacking purine bases, and deaminated cytosines, which are common following a heat treatment (Oei, Vriend, Crezee, Franken, & Krawczyk, 2015). Heat increases the frequency of single-strand breaks (SSBs) and double-strand breaks probably by messing up the restoration of distorted bases. Temperatures above 41 oC, which are present in hyperthermia, instigate the pivotal phosphorylation of histone H2AX, which resembles the creation of ionizing radiation-induced foci that occur following double-stranded breaks in DNA. Hyperthermia encourages DSBs by promoting incisions near conflicting strands of DNA. Moreover, heat promotes chromatin alterations that activate the DNA damage responses without genuine DNA damage.
Hyperthermia also promotes cell death through the instigation of various checkpoint machinery that controls apoptosis. Heat causes DSBs in G1/G2 stages cells as opposed to those in the S-phase. High temperatures impede the advancement of the replication center in these cells. S-phase cells demonstrate increased susceptibility to heat through increased destruction of chromosomes. However, most heat damage is seen in the M-phase, which destroys the cellular mitotic system hence lowering the efficiency of cell division and causing polyploidy. Therefore, it can be concluded that hyperthermia triggers a slow form of death in cells in the S and M phases and rapid death for cells in the G1-stage (Oei et al., 2015).
Therefore, going by the findings of previous studies and the observations of this study, it can be deduced that nanoparticles traversed the nucleus membrane and interacted with chromosomes of cancer cells by imposing heat-induced alterations that affected the DNA of the cancerous cells hence causing their death.
Conclusion
The co-precipitation method was a dependable method of creating magnetite nanoparticles as was evidenced by the creation of nanoparticles whose features were within the published values. Intracellular uptake of the nanoparticles was achieved and ascertained by several techniques including transmission electron microscopy. Prostate cells incubated with high concentrations of nanoparticles showed characteristic motility under the influence of the external magnetic field. Transmission electron microscopy also revealed the presence of nanoparticles within the vacuoles of the cells. It was also evident that the nuclei of prostate cancer cells had a high affinity for the magnetite nanoparticles as was shown by the movement of vacuoles containing the nanoparticles towards the nucleus.
These observations led to the conclusion that magnetite nanoparticles had the capacity to traverse the cell membrane of prostate cells and enter the cytoplasm, particularly the vacuoles and find their way to the nucleus. It was also established that the highest nanoparticle concentration of 50 x 1016 nanoparticles per mL did not promote cell survival. Conversely, low nanoparticle concentrations ranging from 10 x 1016 to 0.5 x 1016 allowed the survival of cells. Additionally, it was observed that the extent of magnetization was proportional to the intake of nanoparticles, which was higher in the cancerous prostate cells than in the normal cells. The motion of the nanoparticles towards the nucleus led to the death of the prostate cancer cells through chromosomal interaction, particularly heat-induced damage to DNA, which affected the cell cycle and led to apoptosis. Therefore, the study concluded that magnetic initiation heating of superparamagnetic nanoparticles to attain restricted hyperthermia in prostate cancer cells had immense remedial promise.
Future Work
The findings of this study showed that magnetic iron oxide led to the death of prostate cancer cells by interacting with the nuclei of cancer cells and interfering with the structure of chromosomes through DNA damage. Also, the cytotoxicity effects were dose-dependent. However, the determination of cell viability of noncancerous cells was obfuscated by the use of trypan blue dye, which complicated the distinction between viable and non-viable cells. Future studies could look into other methods of separating the viable and non-viable cells to provide a clear outlook of the effects of iron oxide nanoparticles on normal cells. This move would help establish the safety of nanoparticles in cancer therapy.
It was reported that the size of coated magnetite nanoparticles was smaller than that of uncoated MNPS (Kumar, Inbaraj, & Chen, 2010; Xiao et al., 2011; Singh, Kim, Patel, Knowles, & Kim, 2012). The reduction in size could be attributed to the presence of surfactants and capping agents, which were known to elevate the steric hindrance between particles (Huynh & Chen, 2011; Niu & Li, 2013). These substances possessed surface ligands with repulsive tendencies, which reduced the extent of particle accumulation and total particle size. Additionally, the saturation magnetization for the coated nanoparticles was reported to be slightly lower than that for the non-coated magnetite nanoparticles (Ranganath & Glorius, 2011; Yang et al., 2013). Some of the substances used to coat MNPs include gum Arabica and sodium citrate. Future studies could look into the effect of magnetic iron oxide coated by sodium citrate on prostate cancer cells. Such a study would elucidate the effect of particle size and saturation magnetization on the chromosomes of prostate cancer cells. These findings could be compared with the present findings and used to determine the attributes that increase the efficiency of magnetite nanoparticles thereby improving the use of magnetite nanoparticles as a potential prostate cancer treatment.
References
Alvarez-Berríos, M. P., Castillo, A., Mendez, J., Soto, O., Rinaldi, C., & Torres-Lugo, M. (2013). Hyperthermic potentiation of cisplatin by magnetic nanoparticle heaters is correlated with an increase in cell membrane fluidity. International Journal of Nanomedicine, 8, 1003-1013.
American Cancer Society. (2016). What are the key statistics about prostate cancer? Web.
Beltran, H., Beer, T. M., Carducci, M. A., De Bono, J., Gleave, M., Hussain, M.,… & Tannock, I. F. (2011). New therapies for castration-resistant prostate cancer: Efficacy and safety. European Urology, 60(2), 279-290.
Estephan, Z. G., Hariri, H. H., & Schlenoff, J. B. (2013). One-pot, exchange-free, room-temperature synthesis of sub-10 nm aqueous, noninteracting, and stable zwitterated iron oxide nanoparticles. Langmuir, 29(8), 2572-2579.
Franke, K., Kettering, M., Lange, K., Kaiser, W. A., & Hilger, I. (2013). The exposure of cancer cells to hyperthermia, iron oxide nanoparticles, and mitomycin C influences membrane multidrug resistance protein expression levels. International Journal of Nanomedicine, 8, 351-363.
García, A., Espinosa, R., Delgado, L., Casals, E., González, E., Puntes, V.,… & Sánchez, A. (2011). Acute toxicity of cerium oxide, titanium oxide and iron oxide nanoparticles using standardized tests. Desalination, 269(1), 136-141.
Gautier, J., Allard-Vannier, E., Munnier, E., Soucé, M., & Chourpa, I. (2013). Recent advances in theranostic nanocarriers of doxorubicin-based on iron oxide and gold nanoparticles. Journal of Controlled Release, 169(1), 48-61.
Glauert, A. M., & Lewis, P. R. (2014). Biological specimen preparation for transmission electron microscopy. Princeton: Princeton University Press.
Guardia, P., Di Corato, R., Lartigue, L., Wilhelm, C., Espinosa, A., Garcia-Hernandez, M.,… & Pellegrino, T. (2012). Water-soluble iron oxide nanocubes with high values of specific absorption rate for cancer cell hyperthermia treatment. ACS Nano, 6(4), 3080-3091.
Heidenreich, A., Bellmunt, J., Bolla, M., Joniau, S., Mason, M., Matveev, V.,… & Zattoni, F. (2011). EAU guidelines on prostate cancer. Part 1: Screening, diagnosis, and treatment of clinically localized disease. European Urology, 59(1), 61-71.
Humphrey, P. A. (2012). Histological variants of prostatic carcinoma and their significance. Histopathology, 60(1), 59-74.
Huynh, K. A., & Chen, K. L. (2011). Aggregation kinetics of citrate and polyvinylpyrrolidone coated silver nanoparticles in monovalent and divalent electrolyte solutions. Environmental Science & Technology, 45(13), 5564-5571.
Jahn, J. L., Giovannucci, E. L., & Stampfer, M. J. (2015). The high prevalence of undiagnosed prostate cancer at autopsy: Implications for epidemiology and treatment of prostate cancer in the Prostate‐specific Antigen‐era. International Journal of Cancer, 137(12), 2795-2802.
Jang, H. J., Lee, J. Y., Lee, D. H., Kim, W. H., & Hwang, J. H. (2010). Current and future clinical applications of high-intensity focused ultrasound (HIFU) for pancreatic cancer. Gut Liver, 4(Suppl 1), 57-61.
Joshi, M., Tiwari, G., Tiwari, R., & Srivastava, B. (2012). Nanomedicine to improve drug delivery outcomes. Chronicles of Young Scientists, 3(4), 258-268.
Kennedy, J. E., Ter Haar, G. R., & Cranston, D. (2014). High intensity focused ultrasound: Surgery of the future? The British Journal of Radiology, 76(909). Web.
Kumar, R., Inbaraj, B. S., & Chen, B. H. (2010). Surface modification of superparamagnetic iron nanoparticles with calcium salt of poly (γ-glutamic acid) as coating material. Materials Research Bulletin, 45(11), 1603-1607.
Laurent, S., Dutz, S., Häfeli, U. O., & Mahmoudi, M. (2011). Magnetic fluid hyperthermia: focus on superparamagnetic iron oxide nanoparticles. Advances in Colloid and Interface Science, 166(1), 8-23.
Levine, G. N., D’Amico, A. V., Berger, P., Clark, P. E., Eckel, R. H., Keating, N. L.,… & Zakai, N. (2010). Androgen‐deprivation therapy in prostate cancer and cardiovascular risk: A science advisory from the American Heart Association, American Cancer Society, and American Urological Association: Endorsed by the American Society for Radiation Oncology. CA: A Cancer Journal for Clinicians, 60(3), 194-201.
Li, K., Zhao, X., K. Hammer, B., Du, S., & Chen, Y. (2013). Nanoparticles inhibit DNA replication by binding to DNA: modeling and experimental validation. ACS Nano, 7(11), 9664-9674.
Lu, J., Liong, M., Li, Z., Zink, J. I., & Tamanoi, F. (2010). Biocompatibility, biodistribution, and drug‐delivery efficiency of mesoporous silica nanoparticles for cancer therapy in animals. Small, 6(16), 1794-1805.
Mahmoudi, M., Azadmanesh, K., Shokrgozar, M. A., Journeay, W. S., & Laurent, S. (2011). Effect of nanoparticles on the cell life cycle. Chemical Reviews, 111(5), 3407-3432.
Monsuez, J. J., Charniot, J. C., Vignat, N., & Artigou, J. Y. (2010). Cardiac side-effects of cancer chemotherapy. International Journal of Cardiology, 144(1), 3-15.
Moyer, V. A. (2012). Screening for prostate cancer: US Preventive Services Task Force recommendation statement. Annals of Internal Medicine, 157(2), 120-134.
Naqvi, S., Samim, M., Abdin, M., Ahmed, F. J., Maitra, A., Prashant, C., & Dinda, A. K. (2010). Concentration-dependent toxicity of iron oxide nanoparticles mediated by increased oxidative stress. International Journal of Nanomedicine, 5, 983-989.
Nefertiti, P. J. (2011). Characterization and evaluation of surface-modified superparamagnetic iron oxide nanoparticles for uptake into human prostate carcinoma cells (PhD). Michigan: University of Michigan.
Niu, Z., & Li, Y. (2013). Removal and utilization of capping agents in nanocatalysis. Chemistry of Materials, 26(1), 72-83.
Oei, A. L., Vriend, L. E., Crezee, J., Franken, N. A., & Krawczyk, P. M. (2015). Effects of hyperthermia on DNA repair pathways: one treatment to inhibit them all. Radiation Oncology, 10(1), 1-13.
Pasticier, G. (2015). High Intensity Focused Ultrasound (Hifu) in Prostate Cancer. In Nargund, V. H., Raghavan, D., & Sandler, H.M. (Eds.), Urological Oncology (pp. 783-798). Springer: London.
Pennycook, S. J., & Nellist, P. D. (Eds.). (2011). Scanning transmission electron microscopy: Imaging and analysis. New York: Springer Science & Business Media.
Petryk, A. A., Giustini, A. J., Gottesman, R. E., Kaufman, P. A., & Hoopes, P. J. (2013). Magnetic nanoparticle hyperthermia enhancement of cisplatin chemotherapy cancer treatment. International Journal of Hyperthermia, 29(8), 845-851.
Ranganath, K. V., & Glorius, F. (2011). Superparamagnetic nanoparticles for asymmetric catalysis—a perfect match. Catalysis Science & Technology, 1(1), 13-22.
Reimer, L. (2013). Transmission electron microscopy: physics of image formation and microanalysis (Vol. 36). New York: Springer.
Roti Roti, J. L. (2008). Cellular Responses to Hyperthermia (40oC-46oC): Cell Killing and Molecular Events. International Journal of Hyperthermia, 24(1), 3–15.
Santoyo Salazar, J., Perez, L., de Abril, O., Truong Phuoc, L., Ihiawakrim, D., Vazquez, M.,… & Pourroy, G. (2011). Magnetic iron oxide nanoparticles in 10− 40 nm range: Composition in terms of magnetite/maghemite ratio and effect on the magnetic properties. Chemistry of Materials, 23(6), 1379-1386.
Sciarra, A., Innocenzi, M., Alfarone, A., Cattarino, S., Gentilucci, A., Minisola, F.,… & Salciccia, S. (2012). Cryotherapy for prostate cancer. European Urological Review, 7(1), 17-23.
Shen, M. M., & Abate-Shen, C. (2010). Molecular genetics of prostate cancer: new prospects for old challenges. Genes & Development, 24(18), 1967-2000.
Silva, A. C., Oliveira, T. R., Mamani, J. B., Malheiros, S. M., Malavolta, L., Pavon, L. F.,… & Martins, M. J. (2011). Application of hyperthermia-induced by superparamagnetic iron oxide nanoparticles in glioma treatment. International Journal of Nanomedicine, 6(3), 591-603.
Singh, R. K., Kim, T. H., Patel, K. D., Knowles, J. C., & Kim, H. W. (2012). Biocompatible magnetite nanoparticles with varying silica‐coating layer for use in biomedicine: Physicochemical and magnetic properties, and cellular compatibility. Journal of Biomedical Materials Research Part A, 100(7), 1734-1742.
Xiao, L., Li, J., Brougham, D. F., Fox, E. K., Feliu, N., Bushmelev, A.,… & Fadeel, B. (2011). Water-soluble superparamagnetic magnetite nanoparticles with biocompatible coating for enhanced magnetic resonance imaging. ACS Nano, 5(8), 6315-6324.
Yang, H. M., Park, C. W., Ahn, T., Jung, B., Seo, B. K., Park, J. H., & Kim, J. D. (2013). A direct surface modification of iron oxide nanoparticles with various poly (amino acid) s for use as magnetic resonance probes. Journal of Colloid and Interface Science, 391, 158-167.
Zhao, H., Halicka, H. D., Li, J., Biela, E., Berniak, K., Dobrucki, J., & Darzynkiewicz, Z. (2013). DNA damage signaling, impairment of cell cycle progression, and apoptosis triggered by 5‐ethynyl‐2′‐deoxyuridine incorporated into DNA. Cytometry Part A, 83(11), 979-988.