Introduction
Background Information
The brain is an integral part of any living organism. The brain receives blood from the heart and this blood contains oxygen and nutrients that nourish it. At the same time, it carries away any metabolic waste that result from breakdown of the nutrients. Of all the organs in the body, the brain receives the bulk of the blood pumped out by the heart accounting for up to 20% of total cardiac output (Deibler 2008).
Conditions that alter the perfusion of brain have wide-ranging implications, eventually resulting to death. Medical conditions such as stroke and cardiovascular accidents are a direct outcome of impaired or defective perfusion of the brain (Detre 2007). Quantification of cerebral blood flow is an essential undertaking in determination of various disease states, prognosis, and assessment of success of treatment (Martin, Friston, Colebatch and Frackowiak 1991).
Cerebral Blood Flow (herein referred to as CBF) is a function of the total metabolic and perfusion functions of the brain at any one given time, whether in the resting or working state. By assessing the blood flow in different areas of the brain, it is possible to extrapolate the results in determining the state of the brain in terms of its function in the respective areas. The measure of perfusion can be made quantitatively by determining the amount of blood circulating in a specific region of the brain (Figueiredo, Clare and Jezzard 2005). Assessment entails factoring the volume of blood delivered to the region under investigation, the size of the region and the time of delivery of blood to the specific region. Imaging of CBF in the paediatric population is a relatively new field, with little research on proper and safer imaging methods available. In the past, application of invasive techniques that entailed the use of radioactive contrast agents was heavily used for pediatric brain imaging and measurement of CBF (Martin et al 1991). Use of these agents in the pediatric population is subject to ethical and moral issues due to the myriad side effects associated with such techniques. For example, it is noted that PET imaging can lead to exposure to radiation on the part of the patient (Figueiredo et al 2005).
Problem Statement
Arterial spin labelling (herein referred to as ASL) has been used successfully in adults to quantify cerebral blood flow (Martin et al 1991). This technique is more accurate and precise as compared to other invasive techniques such as the use of radioactive contrast agents analysed above.
Despite the success recorded in adults, application of ASL in children is limited. This is despite the fact that the few studies that have been conducted in this field points to the fact that it is a feasible technique in the paediatric population (Detre 2007). Arrival time is an important parameter for accurate quantification of CBF in children.
It is against this backdrop that this study was conducted. The current study is a critical review of literature that exists in the field of cerebral blood flow quantification in children. The research is going to pay special attention to arterial spin labelling as a technique in quantification of cerebral blood flow. The topics that will be addressed in this literature review include, among others, an analysis of the invasive techniques in CBF and an analysis of ASL as a non-invasive technique.
Aims and Objectives of the Study
In carrying out this literature review, there were a set of predetermined aims and objectives. These are the goals that the study aimed at achieving from the findings of the literature review. The following are the aims and objectives of this literature review:
- Test a pulsed arterial spin labelling (herein referred to as PASL) technique within a paediatric population
- Make a comparison between a single time point and multiple time point acquisition
- Determine average values for CBF and arrival time in young group
- Compare average values for CBF and arrival time between young and adult populations
- Make recommendations for the use of ASL in children
- Compare the perfusion on pre-cranio and post-cranio surgery
Significance of the Study
The findings of this study will have major implications in the field of CBF quantification, especially in paediatric population. The following are some of the significances of the findings of the study:
- The study will identify the various challenges and limitations of invasive CBF quantification techniques, and help the practitioners in this field in appreciating the non-invasive techniques such as ASL
- From the findings of this study and the recommendations that will be made, practitioners in this field will be able to come up with strategies that will help in realising the full potential of ASL especially in paediatric population
- The study will identify areas that need more research in the future in the field of ASL, and this will be beneficial in this field
Scope and Limitation of the Study
The following are some of the scopes and limitations of this study:
- The study will be limited to the analysis of CBF in children. Adult population, where referred to, will not be the central focus of the study; rather, adult population will be used to build on arguments made in paediatric CBF
- The study will limit itself to the analysis of quantification of CBF using ASL technique. Other techniques such as the use of radioactive contrast agents will not be the central focus of the literature review
- The study will also be limited to the analysis of ASL techniques in paediatric population. This is despite the fact that the technique is also used in adults, and with a lot of success
- The data for the study will be collected from one children hospital, called Alder Hey. This means that the study will be limited to this hospital and the children that will be used in collecting information
Literature Review
Introduction
In chapter one, the study provided the highlights of the study, in preparation for the literature review that will follow. The chapter created awareness on what to follow from the rest of the paper. Among the areas covered in this chapter included the problem statement, significance of the study, study aims and objectives, scopes and limitations among others.
In this chapter, the paper is going to provide the reader with a review of relevant literature in this field. Special attention will be paid to arterial spin labelling in paediatric population.
Invasive Measures of CBF
In earlier methods of determination of brain perfusion, researchers utilized tracers that had the ability to cross the blood brain barrier in and out of the brain. Most of these methods were invasive in nature and they entailed injection of a radiolabelled tracer into the general circulation and monitoring its distribution in the tissues. Agents used included, deuterated water (D2O) and radio-labelled Fluorine gas (Deibler 2008).
Older methods such as Positron Emission Tomography (PET) entailed injection of radioactive water molecules into general circulation. After injection, the tracer would commence its decay by releasing positive charges called positrons. With the help of special machines, the positrons would then be spotted as they circulated and penetrated. The amount of positrons in a given region of the brain is proportional to the amount of blood that specific region receives. Furthermore, other methods such as the use of endogenous tracers like gadolinium-diethyltriaminepentaacetic acid (Gd-DTPA) have been used in quantification of cerebral blood flow in children with brain injury via a process called dynamic contrast-enhanced MRI [DCE-MRI] (Detre 2007).
All of these methods have ethical and moral issues especially when applied to children, hence the need for a method that is non-invasive and safer to use in the paediatric population (Takahashi, Shirane and Sato 1999).
Modern methods of assessing cerebral blood flow utilize the same principles of tracking a labelled compound as it circulates in blood. The difference arises from the non-invasive nature of these modern methods when compared to earlier methods that were invasive. The non-invasive nature of these methods makes them appropriate for use among the paediatric population. An example of such a technique is the use of the arterial spin labelling technique (ASL) in neuroimaging studies (Tofts 2003). This technique is safer for use in children and other patients that maybe affected by exposure to radiation. The subsequent sections of this paper are going to look at ASL within the context of paediatric population.
Arterial Spin Labelling in Children
An Overview
Arterial Spin Labelling (ASL) is one of the modern methods used in determination of cerebral blood flow (CBF). This technique entails the use of tracers just like in the earlier methods only that in ASL the tracer is magnetically labelled rather than radioactively labelled. ASL has been used in determination of perfusion of various body organs such as the kidney, liver among others. The brain is such one organ where ASL is used in determining perfusion, given the fact that the quantity of blood going into the brain is a major determinant of one’s health status (Rusinek et al 2008).
The tracer in ASL is magnetized arterial blood water. The magnetisation of arterial blood water is inverted by a radiofrequency pulse. The magnetized arterial water acts as an endogenous tracer (Detre, 2007). Magnetization of the arterial water is carried out just below the area of interest (slice). The magnetized water, after some time (arrival time) migrates into the area of interest then into the tissues where it exchanges with water in the tissues. The water that is innate to the tissues acquires magnetization from the inflowing labelled water and as such alters the resultant intensity of the image. This image is referred to as the tag or labelled image. Thereafter, the process is repeated but without inversion of the arterial blood water to obtain what is referred to as the control image (Silver and Joseph 1985).
Once the two images are obtained, a calculated subtraction is carried out to produce a perfusion-weighted image. This image represents the amount of blood that is distributed within the particular voxel in the specific slices under a specified arrival time. Subtraction entails pixel-by-pixel elimination process to achieve a true representation of the voxel’s perfusion status (Barbier, Lamalle, and Decorps 2001).
The perfusion-weighted image is in essence a signal difference between magnetized arterial blood water entering the tissue and the tissue water that is unmagnetized. To achieve this perfusion signal, a systematic delay (inversion delay) is initiated between the time of inversion of arterial blood and the time of acquiring the image. The perfusion signal is under the influence of several factors such as the arrival time, CBF and the T1 of the blood and tissue. Due to probable variations in readings and poor signal to noise ratio (SNR), multiple readings are obtained and averaged to boost the SNR.
There are two approaches for Arterial Spin Labelling based on the manner in which the magnetizing signal is used to invert the arterial blood water. These are Pulsed Arterial Spin Labelling (PASL) and Continuous Arterial Spin Labelling (CASL), depending on the mode of application of the radiofrequency pulse.
Approaches for ASL
The following are the two approaches to ASL:
- Continuous Arterial Spin Labelling (CASL)
The basic principle behind the use of CASL entails the application of a radiofrequency pulse that is continuous just below the slice of interest. The frequency follows an adiabatic system that is continuous in nature. As the radiofrequency is applied, a magnetic field gradient is applied parallel to the flow of arterial blood water resulting in inversion of magnetisation of the protons found in the arterial blood water (Zappe et al 2007). In most cases the labelling slice is demarcated from the start of the circle of Willis or close to the common carotid artery and any spin that goes past this point is continuously inverted due to presence of a continuous radiofrequency (Adel et al, 2008).
In CASL, the efficiency of labelling approaches 95% (Buxton et al, 1998). Due to constant magnetization of the spins in CASL, a process called magnetization transfer (MT) occurs which affects the signal in the imaging slices (Buxton et al 1998). A control image is therefore collected without labelling but with matched MT in order to remove the MT effect from the subtraction image.
CASL is a useful method but it is limited due to the extended periods of labelling that are magnified especially when using high magnetic field strengths. Furthermore, the arrival time in CASL is long such that in some disease states there is decay long before the signal reaches the desired voxel resulting in false readings.
- Pulsed Arterial Spin Labelling (PASL)
PASL is a form of arterial spin labelling that entails use of a short radiofrequency pulses to label a large volume of blood. Like other ASL methods, PASL is non-invasive and produces perfusion signals that are under the influence of a myriad of determinants such as arrival time and period width of the specific label. The radiofrequency is usually applied in pulses close to the slice of interest (Calamante, Thomas, Pell, Wiersma and Turner 1999). In the majority of the cases, a hyperbolic secant pulse brings about inversion.
PASL entails three principle methods: EPISTAR, FAIR and QUIPPSS
EPISTAR (Echo Planar Imaging and Signal Targeting with Alternating Radiofrequency)
This involves the utilization of an 180o radiofrequency pulse to facilitate the inversion of the longitudinal magnetization of the protons present in a slab that is below the slice of interest. Following a delay (inversion time), an echo planar image snapshot is taken. The resultant signal is a culmination of labelled arterial blood that is flowing into the slice. The control image is obtained by strategically positioning the slab above the image slice, an area that is devoid of inflowing arterial blood. This is of value in eliminating interference that may occur because of magnetization transfer.
Variation in readings from the different slices may be minimized through application of what is called a saturation pulse just before application of the tag and image pulses (Zhang, Silva, Williams and Koretsky 1995). This aims at minimizing the static magnetization of the tissues under investigation to reduce any interference that might occur during image extraction. The resultant perfusion image is a representation of the extent of perfusion of the voxel under a specified period determined by the predetermined arrival time. STAR is the labelling scheme used in this project to measure CBF.
FAIR (Flow-sensitive Alternating Inversion Recovery)
This is another PASL method that entails the tagging of all arterial blood water external to the region of the imaging slice to minimize the delays in arrival that may result in loss of signal strength. The working geometry of the inversion slab in FAIR is altered to encompass contributions of blood entering into the slice of interest from the top. The pulse used in obtaining the label is non-selective followed by a selective gradient pulse to obtain the control image. Because of this sequence, interferences brought about by magnetizing transfer are completely eliminated (Zoccoli, Lucchi and Andreoli 1996).
Quipss II (quantitative imaging of perfusion using a single subtraction)
This is another PASL technique that has an advantage of versatility since it can be used with almost all labelling geometries. The inverted area is taken through a saturation process immediately after inversion to suppress the end of the inflowing bolus. As such, the time labelled arterial blood water takes to migrate from the plane of labelling to the image slice is shortened. If the image is obtained immediately after the whole of the bolus flows into the image slice, the measurement is no longer sensitive to arrival time. Hence, accurate CBF measures can be made at a single inversion time (Zoccoli et al 1996).
Other forms of PASL
Other forms of Pulsed Arterial Spin Labelling have found use in modern imaging studies. These methods are divided further depending on their symmetry in application.
Asymmetric PASL Methods
They include the Transfer Insensitive Labelling Technique (TILT), Double Inversion with Proximal Labelling of both tagged and control images (DIPLOMA), and Signal Targeting with Alternating Radio frequency – Half-Fourier Single Shot Turbo spin-Echo (STAR-HASTE).
Symmetric Labelling Sequences
These entail the use of methods such as Uninverted Flow Alternating Inversion Recovery (UNFAIR), Flow Alternating Inversion Recovery Extra Radiofrequency pulse (FAIRER), Flow Alternating Inversion Recovery Excluding Radiation damping (FAIRER) and unprepared Basis and Selective inversion (BASE).
Other PASL methods have been named based on the labelling method (Hendrikse, Petersen and Laar 2007). These include, Pulsed Star labelling of Arterial Regions (PULSAR) and Quantitative Star labelling of Arterial Regions (QUASAR). All these methods have found use in advanced modern imaging techniques but most of them have not been studied in the paediatric population (Bode and Wais 1988).
ASL Quantification
According to Petersen et al (2006), conventional CBF quantification is performed using the tracer clearance theory. This theory by Kety and Schmidt (Petersen et al 2006) has been incorporated in CBF quantification using ASL technique. This theory conceptualises inverted arterial blood water as a freely diffusible tracer, and this is its major assumption. According to the theory, there is an instantaneous exchange between the tracer and tissue water when the tracer arrives to the parenchyma (Petersen et al 2006).
ASL quantification requires the availability of data on the exact temporal length of the “bolus of tagged arterial blood” (Petersen et al 2006: p220). This is together with the exact acquisition of the arterial input function (herein referred to as AIF), and thirdly, the tissue curve at high temporal resolution (Wang and Licht 2006).
Physiological mass transport and exchange mechanisms are all functions of ASL quantification. The two are taken as linear stationery systems that can accommodate superposition. Given that it is stationary, there is time-invariance as far as the system’s response to changes in a particular input is concerned (Petersen et al 2006). This is especially applicable in ASL quantification experiments that last some minutes. During such as experiment, it is necessary that no major physiological alterations on the child take place.
With the above parameters in place, it is possible then to calculate tissue perfusion in the child and other patients. Tissue perfusion (designated Ft in the equation below) is computed using the change in the curve of the tissue and arterial input function.
Equation 1: Tissue Perfusion Quantification using ASL
According to Petersen et al (2006), R(t) is the residual function representing the fraction of contrast left in the system after a given time, denoted by t (p. 220).
This model illustrates tracer kinetics utilising a single compartment, and further assumptions are needed to make it work. Such assumptions include the utilisation of “nondispersed square input function or negligible transit time of the tracer” (Petersen et al 2006: p. 219).
The assumptions made in the above model may be valid and applicable in healthy individuals (in this case healthy children). However, the assumptions may not be applicable in pathological cases. They may lead to biased estimation of cerebral blood flow in the child.
Model Errors
Various parameters have errors that are unique to them and these errors have an overall effect on the perfusion readings obtained by use of ASL. The error of propagation elaborates the impact of each parameter on the resultant error in the perfusion measurements. The arrival time can either be measured (using multiple inversion time points) or assumed. Neurological pathologies of the brain such transient ischaemic attacks and stroke (MacIntosh et al, 2008) affect the arrival time such that is important to measure it in these conditions in order to provide accurate CBF measures. To determine arrival time with the highest precision modifications need to be made to the two Arterial Spin techniques. This entails obtaining measurements at different delay times when using CASL. Or different inversion time when using PASL. These two approaches reduce the error due to arrival time differences, but result in elongation of the imaging times (Deibler et al, 2008).
Efficiency of Labelling
Efficiency in labelling plays a major role in determining the success of ASL in the measurement of cerebral perfusion. In CASL, the efficiency in labelling is a function of the speed of blood and the orientation of the labelling plane. This efficiency may be reduced in certain medical pathologies that may result in false readings. The PASL method has a wide labelling area covering a massive capacity of blood vessels even though the length of the labelling slab is limited. As such any arterial blood water outside the labelling slab will not be inverted, a limitation that multiplies when a multislice imaging system is employed since the amount of unlabelled arterial blood water is elevated. Other errors called model errors have an impact on the type of results obtained in determination of cerebral blood flow.
Use of ASL in Pediatric Imaging
As earlier indicated in this paper, Arterial Spin labelling is one of the methods used to measure cerebral blood flow in adults. Since this method is non-invasive, it provides a desirable platform for determining cerebral blood flow without a conflict of moral and ethical aspects encountered in other methods that entail injection of tracers (Chen, Mai, Pippa and Edelman 2001). As such, arterial spin labelling is an appealing method in the paediatric population.
A number of studies have been carried out to determine the efficacy of ASL in children. Wang et al (2002) carried out a study to determine the efficacy and accuracy of arterial spin labelling as a method of determining cerebral perfusion in paediatrics. In this study, the authors set out to determine the longitudinal reproducibility (precision) and accuracy of arterial spin labelling when compared to a phase-contrast MRI, a method commonly used in paediatric populations (Wang and Licht 2006).
By using the two forms of ASL, the researchers found that reproducibility of the results was achieved using the pseudo-Continuous Arterial Spin Labelling (pCASL) when compared to Pulsed Arterial Spin Labelling (PASL) in the paediatric population under study. For example, at 3.0 T, there was a 30 percent to forty percent improvement in SNR produced by the use of AM CASL when compared with PASL (Wang et al 2003: p. 155). Furthermore, the study showed that with an increase in age there is a corresponding increase in accuracy and reproducibility of pCASL. The authors attributed this to un-standardized variables in the paediatric population such as elevated velocity of blood in children as compared to adult populations (Chiron, Raynaud and Maziere 1992). In addition, the positioning of the head during tagging may be the cause of low accuracy observed when using PASL, since in PASL the head position is varied while in pCASL the position is fixed.
Evidence from studies conducted in this field indicates that CBF changes with age. A Study carried out in children and adults that involved mapping of cerebral blood flow patterns showed a distinct difference in perfusion between children and adults especially in the grey matter (Biagi et al 2007). In this study, Continuous Arterial Spin Labelling was used. The study showed a decrease in perfusion among adults when compared to children up to the age of sixteen when there was a rapid drop in cerebral perfusion (Biagi et al 2007). This was attributed to developmental processes occurring in the paediatric population such increased neuron production and myelination that require a constant supply of oxygen and nutrients to facilitate proper and timely development.
Increased perfusion in the grey matter between the children and adults under study was attributed to increased rate of gyrification and synapse formation as one grows older. In this study, other factors such as gender were considered and it was observed that in children, females had relatively lower cerebral perfusion when compared to male children (Biagi et al, 2007). Furthermore, perfusion in the grey matter followed a similar trend in the children. The observed differences were linked to differences in haematocrit concentration between the two genders. In addition, hormonal factors such as presence of oestrogen were implicated in the observed differences (Biagi et al, 2007).
In another study, Pulsed Arterial Spin Labelling at 1.5T was used in determining the cerebral perfusion in children (Wang et al, 2006). The relation of age to values of Signal-to-Noise ratio was assessed in this study. It was observed that the signal was clearer in images obtained by PASL in children than in adults especially in the cortical and sub-cortical areas of the grey matter. The researchers conclude that PASL was a method that was feasible in measuring cerebral blood flow in children with desired precision and Signal to Noise Ratio (Wang et al, 2006). The figure below from this study will clearly illustrate this difference:
sou
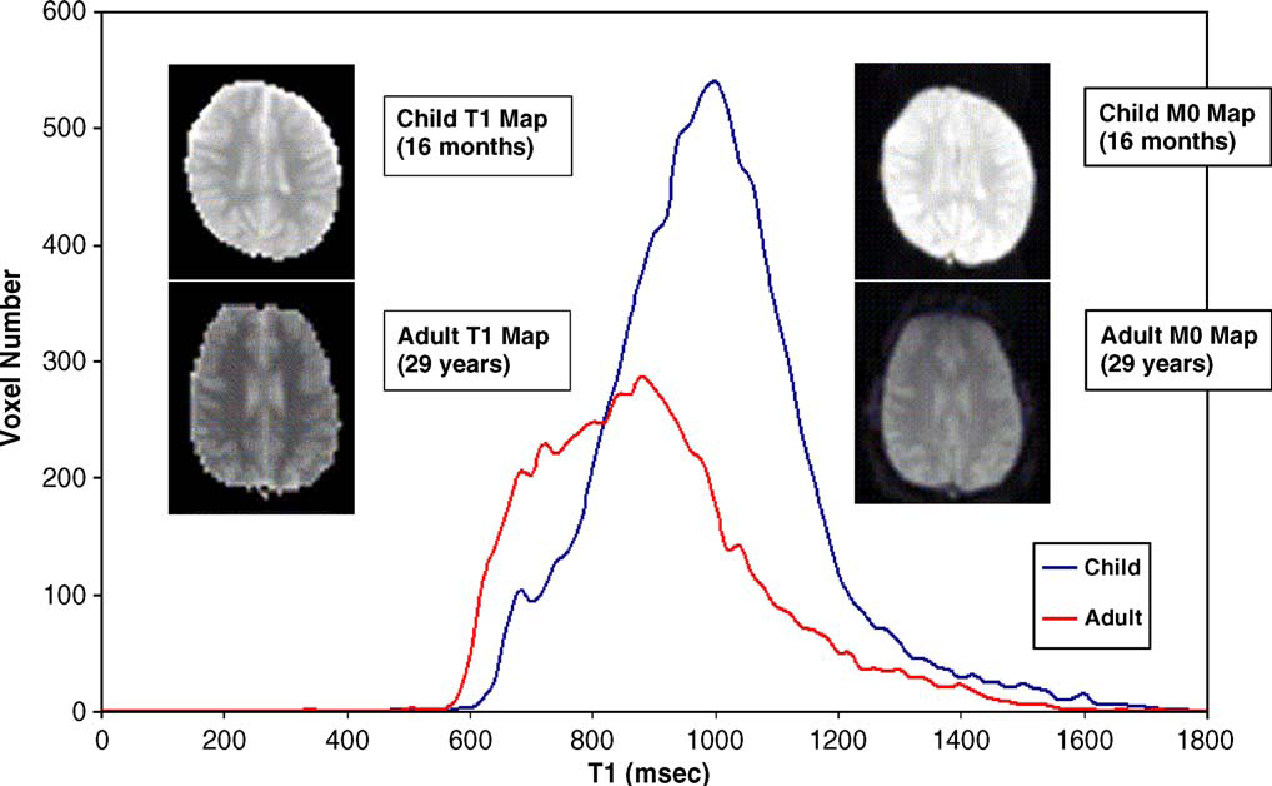
In the graph above, T1 line graph is an average of 7 children and five adults (Wang et al 2006). Representative images T1 and M0 were obtained from a child who was sixteen months old and an adult was 29 years old. The images are displayed using the same scale. It is noted from the above images that those from the child have higher intensities than those obtained from the adult (Wang et al 2006).
Meta-analytic reviews of studies carried out on Arterial Spin Labelling, have compared the two forms of ASL and their applications in modern imaging techniques in adults and children (Miranda, Olofsson and Sidaros 2006). One review compared PASL and CASL in children in terms of a myriad of affecting factors (Petersen, Zimine Golay 2006). Arterial transit time was identified as one of the quantification errors that occur during determination of cerebral blood flow using PASL. As such, modifications such as QUIPSS II which is insensitive to arrival time were recommended. Other errors identified by the review include vascular artefacts, errors in the shape of inversion pulse and its frequency, the partition coefficient of the blood brain barrier and errors of equilibrium magnetization in the different regions of the brain (Petersen, Zimine and Golay 2006).
Some Challenges in the use of ASL
For a clear understanding of the working principles behind ASL, researchers have carried out studies in non-human primates using the two-compartment method to simulate cerebral blood flow. In one study carried out, researchers fitted ASL data obtained in monkeys in a two-compartment model under different physiological conditions (Zappe et al, 2007). Furthermore, any expected uncertainties were identified using the Monte Carlo simulations. The researchers found out that inherent errors were associated with the use of ASL in determination of cerebral blood flow. In addition, it was demonstrated that ASL is superior in determination of cerebral blood flow especially in the occipital lobe of the monkey as compared to the use of positron emission tomography (PET). There was a discrepancy in the results obtained when compared to other studies, and this is enough reason to encourage further studies in the use of modified ASL and two-compartment model in determination of cerebral blood flow in primates and humans alike (Zappe et al 2007).
Other reviews of ASL have focussed on the applications of ASL in diagnostic purposes for the various conditions and associated sources of error. One review identified transit delay and incorporation of signal emanating from blood in the intravascular compartment (Wong, Buxton and Frank 1999). In addition, the authors reviewed current solutions to the problem, which include fine-tuning of CASL and OPASL to eliminate these interferences through modifications such as the (quantitative imaging of perfusion using a single subtraction). Furthermore, the authors reviewed the use of arterial spin labelling in measurement of cerebral blood flow under normal and pathologic conditions. The review entailed assessment of efficacy of Arterial Spin Labelling, in determination of cerebral blood flow in stroke patients. The authors identified flaws in application of ASL in such pathologies, since CBF values obtained using ASL were observed to be decreased as a result of elongated transit time. As such, modifications of ASL were identified to be applicable in determining cerebral blood flows in stroke patients (Wong, Buxton and Frank, 1999).
Just like all imaging techniques, ASL has constraints that limit its application in different situations. The diversity in composition of the voxel tissue has an effect on the quantification of cerebral blood flow when using Arterial Spin Labelling. This occurs as a result of effects of partial volume and errors in that occur in conversion of raw ASL signal to Cerebral Blood Flow units. One study revealed that heterogeneity had a major impact on the results obtained from the cortical regions of the brain but there was little change in the results obtained in the deep white matter (Kim 1995). The researchers concluded that correction of the result for partial volume effects results is important.
Even though ASL has received wide acceptance among the radiological and imaging community, it still has issues with standardization and reproducibility especially when used in multicentre studies. This is another challenge that faces the application of this technique in quantification of CBF in children. The lack of reproducibility stems from a myriad of factors that are either intrinsic or extrinsic to the ASL system.
Factors such as low Signal to Noise Ratio, equilibration in blood magnetization, and complications in quantification may affect reproducibility of ASL (Tofts 2003). Furthermore, instrumentation errors such as shimming, improper modulation of the RF signal and human errors such as improper manipulation of the ASL system result in false readings of the cerebral blood flow in determination of cerebral perfusion in children. Studies have been carried out to ascertain the reproducibility of ASL (Parkes, Rashid, Chard and Tofts 2004). This allows for accurate diagnosis, consistent patient follow up, and seamless interpretation of data on perfusion wherever and whenever with consistent results.
Gevers et al (2009) carried out a study to assess the reproducibility of CASL and pCASL in multicentre settings and to ascertain whether the obtained results would be extrapolated in other centres as standards of CBF measurements (Tofts, 2003). They did this by subjecting similar individuals to repetitive scanning using the two techniques in various centres. The researchers observed that pCASL had the highest reproducibility in multicentre while CASL had higher reproducibility in the intracentre. In addition, they observed that in the presence of background suppression, the results were more reproducible. They concluded that intracenter reproducibility was more feasible than multicentre one (Chugani 1998: Luh et al 2000).
The use of ASL on other Body Organs apart from the Brain
This project is looking at the use of ASL in the brain, but it is important to look how the technique has been used in other body organs apart from the brain. This will help in appreciating the applicability of ASL outside the brain, and it will help in identifying some of the challenges that are faced by this technique in these other organs (Alsop et al 2000: Bokkers et al 2008).
Kidneys are the most perfused organs in the body and quantification of blood in these organs is of medical importance since it gives an insight on the origin of a myriad of medical conditions such as hypertension, acute renal failure and diabetes associated nephropathy. One study carried out by researchers set out to quantify blood flow into the kidneys of the rat using ASL. Imaging methods such as the use of gadolinium-diethyltriaminepentaacetic acid (Gd-DTPA) are not feasible in such studies since these tracers are filtered by the renal tubules (Rajendran et al 2005: Robson et al 2009). The researchers observed regional variations in blood flow with highest values being recorded in the cortex while the medulla had little perfusion. Since, no signal was observed in post mortem rats the observed signal was due to renal perfusion (Rajendran, Yong, Tan, Wang and Chaung, 2005). Further evidence was obtained by initiating dilatation of the vessels by administration of CO2 and O2 in a ratio of 1:19 to reduce the transit time and elevated perfusion. The authors employed Matlab in generation of maps of arterial transit time and perfusion in regard of stepwise removal of FAIR images. The researchers observed a change in the signal when using FAIR ASL that was attributed to suspected sensitivity of the method to renal perfusion (Kim, 1995). When the results obtained were compared to available literature, the results tallied implying a feasibility of this method in determination of renal perfusion in humans (Wong et al 2000: Yang et al 2000).
Modifications in ASL have resulted in a wide application of this technique. Techniques such as EPISTAR, STAR-HASTE and FAIR have undergone modification to incorporate more measurements (Robson et al, 2009). A study combining ASL with cardiac-triggered segmented true-FISP, a sequence was acquired and used to develop two methods of quantification namely STAR-TrueFISP (True Fast Imaging with Steady-state Precession) and FAIR-TrueFISP (FAIR-True Fast Imaging with Steady-state Precession). These two had a very high a temporal resolution. The image was acquired through the true-FISP sequencing (Rusinek et al 2008). This method, according to the researchers provided very high resolution and had short TR and TE that allows for rapid imaging process. With such properties, the method can be used in determination of a variety of vascular diseases such as emboli in the pulmonary branch and renal artery stenosis in both adults and children (Ogawa, Sakurai and Kayama 1989). The authors observed setbacks in this method, since; a breath-holding delay is required to minimize interference by chest wall components. Other studies have employed the use of True-FISP but at higher t values. One study used this method at 3T to determine blood flow in the brain’s hippocampus region (Wang et al 2003: Warmuth et al 2003). This region is implicated in various neurological conditions such as epilepsy, Alzheimer’s and Huntington’s disease (Alsop, Detre, and Grossman, 2000). The researchers observed that ASL was applicable in determination of regional cerebral blood flow and as such, it can be used in monitoring of progress of temporal lobe neurological conditions such as Alzheimer’s disease and epilepsy (Chen, Mai, Pippa and Edelman 2001).
Cranio Surgery in Children
The skull is not made up of a single mass of bone enclosing the brain and other internal organs. To the contrary, it is made up of bones that are separated by sutures, as indicated in the diagram below. The diagram depicts the various sutures that are involved in the composition of a skull:
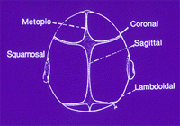
When the child is growing up, the open sutures of the skull are flexible and they allow it to expand, giving the child a normal cranial shape. However, there are cases where the sutures close early, making the skull of the child to expand perpendicular to the closed suture. This leads to an abnormal shape of the head, which may lead to other complications such as reduced perfusion in the brain. Other complications are Crouzon and Apert syndromes, which are the two most common types of craniosynostosis. The latter is the premature closing up of the sutures in the child’s skull.
The complications coming with premature closure of the sutures necessitates operation on the skull. This is referred to as cranio facial surgery, a procedure that is especially used to reduce the pressure on the brain exerted by the malformed skull.
After opening up the skull and carrying out the correctional procedures, there are several techniques used to hold the bones together after they are moved into position. This includes the use of metal plates and screws to make the skull bones remain in place after surgery. However, this technique has various shortcomings especially after subsequent growth takes place in the skull. For example, it has been found that the screws usually poke on the brain, given the fact that the plates usually remain inside the skull. Other techniques may involve the use of dissolving stitches to hold the bones in place, as opposed to using the metal screws and plates. The use of dissolving stitches ensures that there is nothing foreign that is left inside the skull when the child is growing up. A third technique, and which is one of the most recent, is the use of dissolving plates and screws as opposed to the use of the metallic ones. This, like the use of dissolving stitches, ensures that there is nothing foreign that is left inside the skull.
There are several elements that are involved in the cranio facial surgery procedure. These elements are intended to normalise the cranial vault, which is the major aim of the surgery. One of the elements is the minimisation of blood loss during the procedure. This is achieved by injecting vasoconstrictive agents such as epinephrine a few minutes before the skull is opened up. Also, modern surgeons try to avoid the use of titanium plates while fixing the skull. This is given the fact that the plates are gradually displaced towards the brain if the plates are used, as indicated above. This is brought about by the resorption of the innermost bone layer of the child’s skull, accompanied by deposition of new bone on the outermost layer of the skull. This is the reason why most practitioners make use of the absorbable plates so that they do not put the brain in jeopardy.
In the preceding section, the craniofacial surgery in children within the context of children will be looked into. This is especially within the context of cranial blood quantification and craniosynostosis.
Craniofacial Surgery in Children and Cerebral Blood Flow
There are several conditions in children that interfere with cerebral perfusion, or flow of blood in the brain. One such condition is the premature fusion of multiple cranial sutures in children. According to Biagi et al (2007), this condition raises the intracranial pressure, reducing perfusion in the brain. This condition, if not corrected early enough, may lead to mental impairment in the child in later life.
Cranial sutures play a significant role in the development of the child. According to Bode and Wais (1988), the sutures make it possible for the craniofacial skeleton to make adjustments as the child grows. This is in size, shape and spatial bony orientation, factors that are crucial for perfusion in the brain. As the brain develops, it exerts pressure on the calvarial bones, a process that stretches the fibrous suture bands and enlargement of the sutural space (Detre 2007). This means that the sutures are not the site for primary cranial and brain growth and development (Detre 2007). To the contrary, sutures are for adaptation during growth and development.
Complications during growth involve the failure of the suture to form completely, or having the suture obliterated by bone before complete development and cranial adaptation takes place (Detre 2007). This condition may lead to a condition that Biagi et al (2007) refer to as craniosynostosis. Complex or multiple sutures synostosis has been noted to increase intracranial pressure in the child, affecting perfusion and cranial blood flow.
Craniofacial surgery is one of the procedures that are commonly used to correct this condition. In a study cited by Bokkers et al (2008), seven children with ranging levels of craniosynostosis were operated on to correct their conditions. Cranial perfusion of the children was analysed before and after the surgery to see the difference. Single positron emission computed tomography (SPECT) was utilised to analyse the varying cerebral perfusion in the regions that were affected by the sutures. This was done before and after the cranial reconstructive surgery was carried out (Bokkers et al 2008).
In the study, it was found that asymmetries in cerebral blood flow before the craniofacial surgery varied between 0 to thirty percent, with a mean of 13 percent. It was noted that majority of the patients (5 children) with asymmetric cerebral perfusion improved. The perfusion became symmetric after the craniofacial surgery, and no further defects were detected in the follow-up studies.
The study cited above revealed that craniosynostosis decreases cerebral perfusion due to elevated cranial pressure. Craniofacial reconstruction can be used to address this problem, normalising cerebral perfusion and arresting future and possible mental complications in the child.
Chapter Summary
This chapter looked at some of the literature that exists in the field of cerebral blood flow quantification in children. The aim of the literature review was to identify the status of research in this field, and to identify the various consensus and controversies in existence. The researcher looked at the various types of ASL that are used in this field, and how they are applicable to the paediatric patient.
Conclusion
This paper was a critical review of literature that is to be found within the ASL field, with special attention paid to the use of ASL in children. In the literature review, the researcher identified the various forms of ASL techniques that are in use in the contemporary medical field, together with their applicability in the paediatric population. Some of the challenges facing the use of ASL were identified, and recommendations given where necessary on how to deal with these challenges.
References
Adel, J., Sherma, A., Carroll, T., Hage, Z., Miller, J., Walker, M., Hunt, H., et al 2008.
The use of quantitative magnetic resonance perfusion for assessment of CBF in the perioperative management of carotid stenosis: Case illustration. The Open Neurosurgery Journal, 1, 1-5.
Alsop, C., Detre, J., and Grossman, M 2000. Assessment of cerebral blood flow in Alzheimer’s disease by spin-labelled magnetic resonance imaging. Annals of Neurology, 47, 93–100.
Barbier, L., Lamalle, L., and Decorps, M 2001. Methodology of brain perfusion imaging. Journal of Magnetic Resonance Imaging, 13, 496–520.
Biagi, L., Abbruzzese, A., Bianchi, C., Alsop, C., Del Guerra, A., and Tosetti, M 2007.
Age dependence of cerebral perfusion assessed by magnetic resonance continuous arterial spin labelling. Journal of Magnetic Resonance Imaging, 25(1), 696–702.
Bode, H., and Wais, U 1988. Age dependence of flow velocities in basal cerebral arteries. Archives of Disease in Childhood, 63, 606–611.
Bokkers, P., Laar, J., and Ven, C 2008. Arterial spin-labelling MR imaging measurements of timing parameters in patients with a carotid artery occlusion. American Journal Neuroradiology, 29, 1698 –1703.
Buxton, R., Frank, L., Wong, C., Siewert, B., Warach, S., and Edelman, R 1998. A general kinetic model for quantitative perfusion imaging with arterial spin labelling. Journal of Magnetic Resonance Imaging, 40(3), 383-396.
Calamante, F., Thomas, L., Pell, S., Wiersma, J., and Turner, R 1999. Measuring cerebral blood flow using magnetic resonance imaging techniques. Journal of Cerebral Blood Flow and Metabolism, 19(7), 701-735.
Chen, Q., Mai, V., Pippa, S., and Edelman, R 2001. Dynamic ASL flow imaging with cardiac triggered true FISP acquisition. International Society for Magnetic Resonance in Medicine, 9, 1955-1956.
Chiron, C., Raynaud, C., and Maziere, B 1992. Changes in regional cerebral blood flow during brain maturation in children and adolescents. Journal Nuclear Medicine, 33, 696 -703.
Chugani, T 1998. Biological basis of emotions: Brain systems and brain development. Paediatrics, 102, 1225–1229.
Deibler, A., Pollock, J., Kraft, R., Tan, H., Burdette, J., and Maldjian, J 2008. Arterial spin labelling in routine clinical practice. American Journal of Neuroradiology, 29, 1428-1435.
Detre, J 2007. Arterial spin labelled perfusion MRI. Journal of Neurotherapeutics, 4(3), 346–359.
Figueiredo, P., Clare, S., and Jezzard, P 2005. Quantitative perfusion measurements using pulsed arterial spin labelling: Effects of large region-of- interest analysis. Journal of Magnetic Resonance Imaging, 21, 676–682.
Gevers, S., Osch, J., Hendrikse, J., Bokkers, R., Kies, D., and Teeuwisse, W 2009.
Multicentre reproducibility of continuous, pulsed and pseudo-continuous arterial spin labelling: Can we use general reference values of cerebral blood flow? International Society for Magnetic Resonance in Medicine, 17, 626-630.
Hendrikse, J., Petersen, T., and Laar, J 2007. Cerebral border zones between distal end branches of intracranial arteries: MR imaging. Radiology, 246, 572–580.
Kim, S 1995. Quantification of relative cerebral blood flow change by flow sensitive alternating inversion recovery (FAIR) technique: application to functional mapping. Magnetic Resonance Medicine, 34, 293–301.
Luh, W., Wong, E., and Hyde, J 2000. Arterial spin labelling localizes the fMRI signal to brain tissues better than BOLD. Journal of Neuroimaging, 12(4), 442-456.
MacIntosh, B., Lindsay, A., Kylintireas, I., Kuker, W., Gunther, M., Robson, M., et al 2008. Multiple inflow pulsed arterial spin-labelling reveals delays in the arterial arrival time in minor stroke and transient ischemic attack. American Journal of Neuroradiology, 31, 1892-1896.
Martin, J., Friston, J., Colebatch, G., and Frackowiak, J 1991. Decreases in regional cerebral blood flow with normal aging. Journal of Cerebral Blood Flow Metabolism, 11, 684–689.
Miranda, J., Olofsson, K., and Sidaros, K 2006. Non-invasive measurements of regional cerebral perfusion in preterm and term neonates by magnetic resonance arterial spin labelling. Journal of Paediatric Research, 60(3), 359-363.
Ogawa, A., Sakurai, Y., and Kayama, T 1989. Regional cerebral blood flow with age: Changes in rCBF in childhood. Neurology Research, 11, 173–176.
Parkes, L., Rashid, W., Chard, T., and Tofts, P 2004. Normal cerebral perfusion measurements using arterial spin labelling: reproducibility, stability, and age and gender effects. Magnetic Resonance in Medicine, 51(1), 736–743.
Petersen, E., Zimine, I., and Golay, X 2006. Non-invasive measurement of perfusion: A critical review of arterial spin labelling techniques. The British Journal of Radiology, 79, 688–701.
Rajendran, R., Yong, C., Tan, J., Wang, J., and Chaung, K 2005. Quantitative mouse renal perfusion imaging using arterial spin labelling, International Society for Magnetic Resonance in Medicine, 16, 456-460.
Robson, M., Madhuranthakam, J., Dai, W., Pedrosa, I., Rofsky, M., and Alsop, C 2009. Arterial spin labelling. Magnetic Resonance in Medicine, 61, 1374-1375.
Rusinek, H., Brys, M., Switalski, R., Haas, F., Mcgorty, K., Leon, M., et al 2008. Hippocampal blood flow and vascular reserve: TrueFisp ASL at 3T Proc. International Society for Magnetic Resonance in Medicine, 16, 1918-1919.
Silver, M., and Joseph, I 1985. Selective spin inversion in nuclear magnetic resonance and coherent optics through an exact solution of the Bloch-Riccati equation. Physical Review, 31, 2753-2755.
Takahashi, T., Shirane, R., and Sato, S 1999. Developmental changes of cerebral blood flow and oxygen metabolism in children. American Journal of Neuroradiology, 20, 917–922.
Tofts, P 2003. Quantitative MRI of the brain: Measuring changes caused by disease. Chichester: John Wiley and Sons.
Wang, J., Alsop, D., Li, L., Listerud, J., Gonzalez, J., Schnall, M., et al 2002. Comparison of quantitative perfusion imaging using arterial spin labelling at 1.5 and 4.0 tesla. Magnetic Resonance in Medicine, 48, 242–254.
Wang, J., and Licht, D 2006. Paediatric perfusion MR imaging using arterial spin labelling. Journal of Neuroimaging Clinics of North America, 16, 149–167.
Wang, J., Licht, D., Jahng, D., Liu, C., Rubin, J., Haselgrove, J., et al 2003. Paediatric perfusion-imaging using pulsed arterial spin labelling. Journal of Magnetic Resonance Imaging, 18, 404–413.
Warmuth, C., Gunther, M., and Zimmer, C 2003.Quantification of blood flow in brain tumours: comparison of arterial spin labelling and dynamic susceptibility weighted contrast-enhanced MR imaging. Radiology, 228, 523–532.
Wong, E., Buxton, R., and Frank, L 1999. Quantitative perfusion imaging using arterial spin labelling. Neuroimaging Clinics of North America, 9(2), 333-340.
Wong, E., Luh, W., and Liu, T 2000. Turbo ASL: Arterial spin labelling with higher SNR and temporal resolution. Magnetic Resonance in Medicine, 44, 511–515.
Yang, Y., Engelien, W., Xu, S., Gu, H., Silbersweig, D., and Stern, E 2000. Transit time, trailing time, and cerebral blood flow during brain activation: Measurement using multislice, pulsed spin-labelling perfusion imaging. Magnetic Resonance in Medicine 44, 680–685.
Zappe, A., Reichold, J., Burger, C., Weber, B., Buck, A., Pfeuffer, J., et al 2007. Quantification of cerebral blood flow in nonhuman primates using arterial spin labelling and a two-compartment model. Magnetic Resonance Imaging, 25, 775–783.
Zhang, W., Silva, A., Williams, D., and Koretsky, A 1995. NMR measurement of perfusion using arterial spin labelling without saturation of macromolecular spins. Magnetic Resonance in Medicine, 33(3), 370-376.
Zoccoli, G., Lucchi, L., and Andreoli, E 1996. Arterial spin labelling. Journal of Cerebral Blood Flow Metabolism, 16, 1312–1318.